Figure 3 shows that
as the basis set increases, potential energy decreases.
Carbon Monoxide:
The RHF6-21G(d,p) level of theory was selected as
the best level of theory for optimization based on the comparison of
experimental bond length to those calculated from the NIST
website. Figure 4 shows optimized geometry.
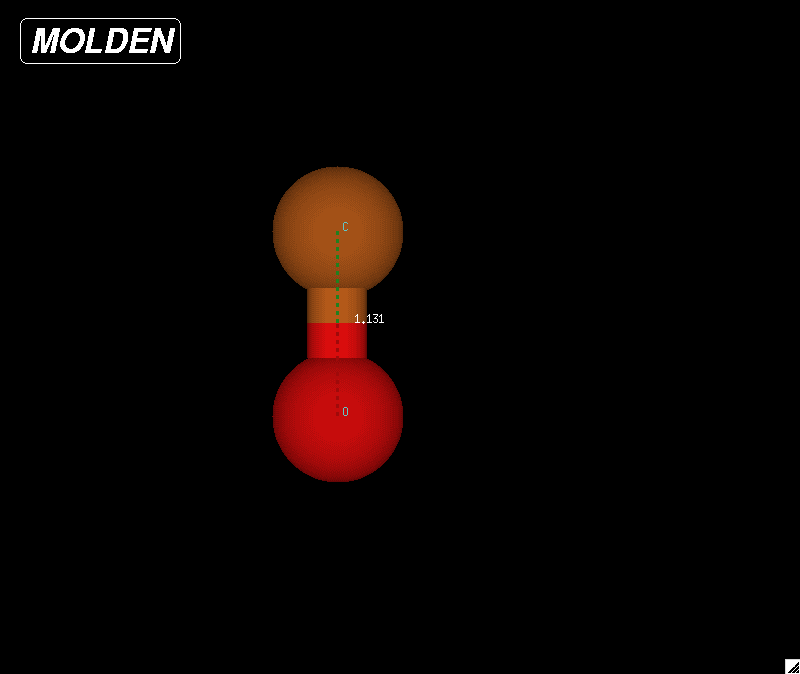
|
Figure 4: Optimized geometry for
a carbon monoxide molecule. The
bond length was determined to be 1.13059 Angstroms based on these
calculations. The NIST website reported a bond length of 1.1283
Angstroms |
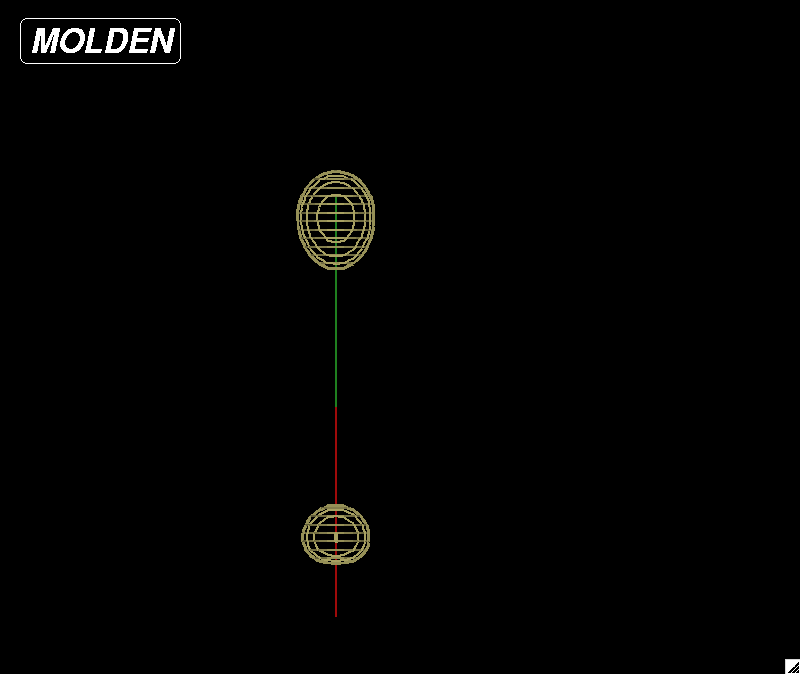
|
Figure 5: HOMO (highest occupied
molecular orbital) for a carbon monoxide molecule. |
According to the
RHF6-21G level of theory, the vibrational frequency was determined
to be 2295.80 1/cm for the stretching vibration between the two
atoms. The NIST website reported a frequency of 2170 1/cm.
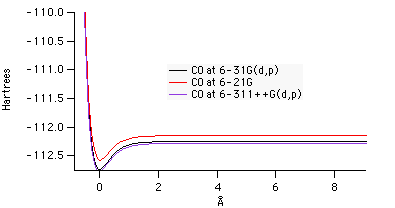
|
Figure 6: Potential energy
combined plot at various levels of theory. |
Figure 6 shows that as the basis set
increases, potential energy decreases. By comparing the plots of
potential energy for fluorine and carbon monoxide, one can see that the
energy is higher for carbon monoxide at each corresponding level of
theory. As energy increases, stability decreases, so these
results imply that fluorine is a more stable diatomic than carbon
monoxide.
Benzoic Acid:
The RHF6-311G(d,p) level of theory was selected as
the best level of theory for optimization based on the comparison of
experimental bond length to those calculated from the NIST
website. Figure 7 shows optimized geometry.
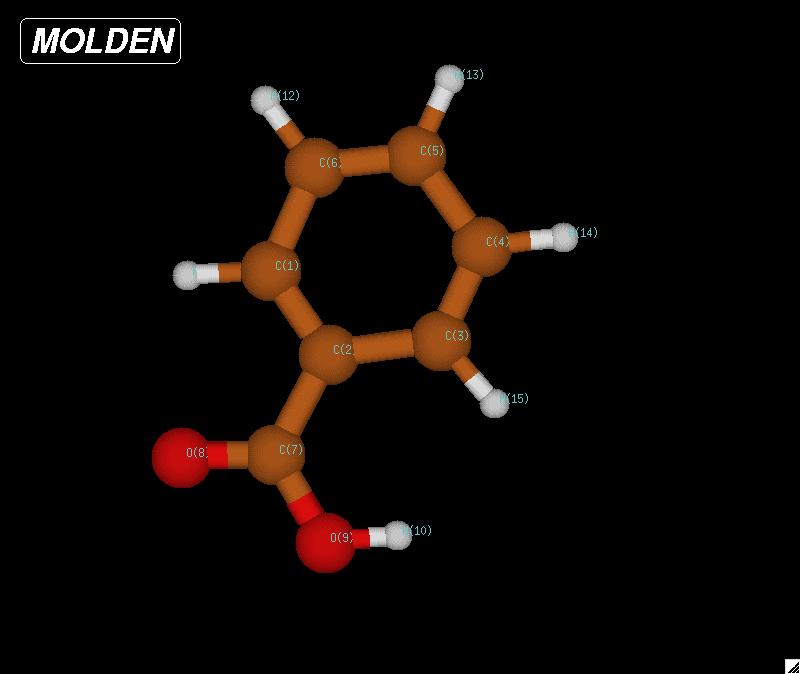
|
Figure 7: Optimized geometry for
a benzoic acid molecule. The
bond lengths were determined to be 1.08977 Angstroms for C-H, 1.33631
Angstroms for C=C based on these
calculations. The CRC Handbook reported a bond length of 1.084
Angstroms for C-H, 1.395 Angstroms for C=C.
|
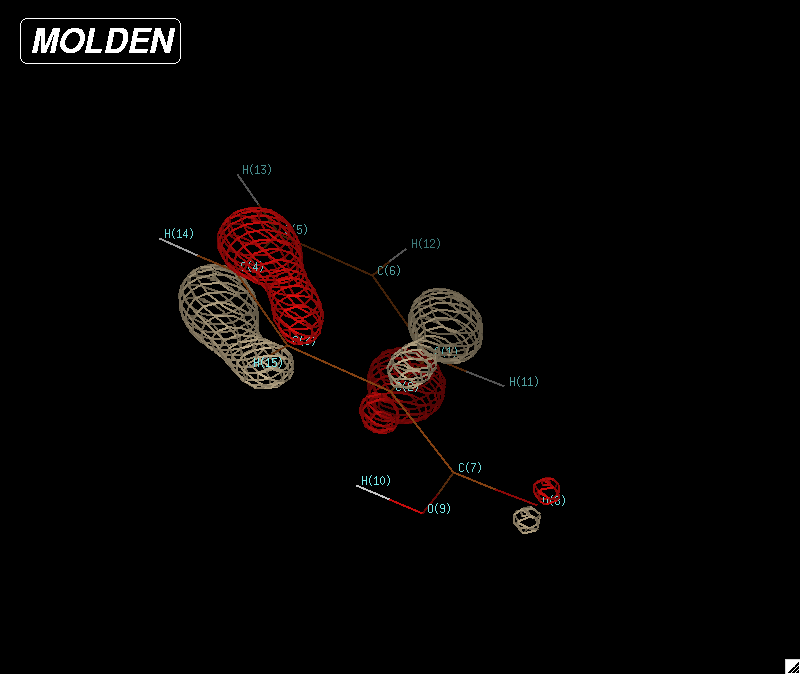
|
Figure 8: HOMO (highest occupied
molecular orbital) for a benzoic acid molecule. |
According to the
RHF6-311G(d,p) level of theory, the vibrational frequency are shown in
the following table.
Calculated
Vibrational Frequencies (1/cm)
|
NIST Vibrational Frequencies
(1/cm)
(From IR Spectrum)
|
C-H:
|
C-H:
|
3780
|
3200
|
3805
|
3000
|
3819
|
2950
|
3827
|
2800
|
3847
|
2650
|
Carbonyl:
|
Carbonyl:
|
2392
|
1730
|
Aromatic Ring:
|
Aromatic Ring:
|
1929
|
2000
|
1905
|
1900
|
O-H wave:
|
O-H wave:
|
1096
|
900
|
Table 1: Shows the calculated vibrational frequencies for different
atom bonding for benzoic acid and compares the calculated values to
values shown on the IR spectrum from the NIST website, the IR
spectrum can be found at the following link:
http://webbook.nist.gov/cgi/cbook.cgi?ID=C65850&Units=SI&Type=IR-SPEC&Index=0#IR-SPEC
6-21 & 6-31 Transition energy (nm)
|
6-21& 6-31 Oscillator
Strength
|
202.6
|
0.001445
|
195.3
|
0.031782
|
189.6
|
0.072450
|
147.4
|
1.109531
|
144.6
|
0.833347
|
134.2
|
0.000008
|
131.3
|
0.000464
|
128.1
|
0.288010
|
126.3
|
0.000369
|
124.2
|
0.008856
|
Table 2: Shows the UV-Vis calculations using the 6-21 and 6-31
levels of theory. The data was compared to a UV-Vis
spectrum from the NIST website. It was noticed that the
calculated data fit into the same relative shape of the spectrum,
however, the calculated excited energy states were too high. To
see the NIST spectrum log on to:
http://webbook.nist.gov/cgi/cbook.cgi?ID=C65850&Units=SI&Mask=400#UV-Vis-Spec
Conclusion:
The programs used in this experiment were
useful in determining information that would be very difficult to
calculate by hand. Computers also have the luxury of being
able to use large basis sets and perform calculations on complex
molecules. Unfortunately, data reported does not always provide
very accurate results, which can be seen in our calculations
above. Computer simulations are useful for student laboratory,
but not reliable for industrial laboratory type settings.
References:
Gutow. "Molecular Orbitals Experiment". 9/05. Pg. 1
NIST website
Lide, D.R. The CRC Handbook of Chemistry and Physics. 81ed. New York:
CRC Press, 2000.
Brooke_Cassidy/371_Protected/M01_Lab